There is something quite “evil genius” about shooting an electron beam with a laser, especially for non-physicists like me. That this causes gamma rays to emerge (back towards the laser) is perhaps even more interesting. Allow me to elaborate in slightly more scientific terms.
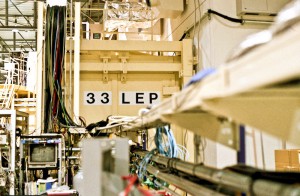
Around the SPring-8 synchrotron are some extraordinary developments, and one of these is a unique high-energy physics experiment. At this beamline, gamma rays (with energies of up to 3 GeV) are generated through Compton backscattering of laser photons against the ring electrons. This is likened to reflection of the laser photons against the electrons, which due to their speed, return these photons at significantly higher energy than that at which they entered.
One way of understanding why a laser photon is reflected and exits as a gamma ray (a photon of much higher energy), is to consider relativistic theory, in particular to be able to move from a “laboratory frame of reference” where you assume that the world is standing still and the electron bunch is moving, to the electron frame of reference, where the electron is standing still and the world is moving. The transformation from the one into the other is called a Lorentz transformation.
In the experiment, starting from a laboratory frame of reference, the photon is transformed into the electron reference frame, and in this Lorentz transformation, gains four orders of magnitude (a factor of about 10000) in energy. Then the photon is reflected (described as a sort of “trick” by dr. Daté) and upon returning to the laboratory reference frame, gains another factor of 10000 in energy. Thus, a total of eight orders of magnitude in energy are gained. One laser on site is a solid-state UV laser with a wavelength around 266 nm, and thus a photon energy of about 4.7 eV. This will then return to the laser with an energy on the order of GeV (in fact with a maximum of around 3 GeV)!
In summary, the photon energy coming out is proportional to the photon energy going into the electron beam. On the other hand, since the Lorentz factor is proportional to the electron energy, the photon energy coming out is proportional to the energy of the electron beam squared. So the higher energy electron rings have a big advantage here (SPring-8 has an 8 GeV ring energy). So why not go to one of the high-energy physics facilities, where the ring energy can be much higher?
One of the reasons for building the experiment at SPring-8 instead of at higher-energy physics facility was that the beam availability at SPring-8 is so much better. Given the low interaction probability (small scattering cross-section) between the gamma photons and some material, the experiments take a long time and therefore a good beam availability is required. Additionally, the energy of the photons just exceeds the threshold to produce a pair of kaons (a strange and anti-strange meson).
These gamma rays open up a variety of research fields, such as the hunt for the disputed “pentaquark”, the investigation into the obscure production mechanism of mesons (a type of subatomic particle), and the search for yet unseen resonance states of baryons. The gamma ray energy implies a wavelength of less than 0.1 femtometer (fm). Too short to see the structure of a nucleus (about 10 fm) or a nucleon (about 1 fm), these are instead ideal for investigating the motions of quarks.
At the other end of the beamline lies the detection system, capable of detecting a variety of charged particles (using magnetic separation and time-of-flight (TOF) to determine the mass/charge ratio of the particles to identify them). This detection system currently consists of a backscattering detector, a trigger counter (for time-of-flight determinations), a powerful (1T) magnet to separate the charged particle trajectories, a few drift chambers to trace particle paths, and finally a large array of 2D TOF counters (c.f. Sumihama et. al., Phys. Rev. C73, 035214 (2006)). These systems require large amounts of power on one end, and an impressive amount of wiring on the other end. It certainly is in stark contrast to your average beamline detector in scale and scope, and the next version will be even more impressive.
THE PENTAQUARK STORY
First observed in 2003 (see f.ex. Nakano et. al., Phys. Rev. Lett. 91, 2003, 012002), the so-called “pentaquark” particle (decaying into a kaon particle and a neutron) has stirred up some physics-dust. The existence of the pentaquark would influence hadron physics considerably, and thus is of interest to many groups. About half of the experimental groups confirmed the existence of pentaquarks, whereas the other half found nothing. The latter includes prominent institutes like Fermilab and Jefferson Lab, strong in the US. Since they have claimed the pentaquark does not exist, the research into its existence is now mainly performed in asia.
There are some hypotheses as to why the particle was only observed by a few groups, having to do with the incident energies, detection systems and production method. Groups such as one at Fermilab search highly excited states in forward directions (with high energies). However, a particle consisting of a large number of quarks (5 for the pentaquark) is hardly produced in this energy region as all quarks must have the same momenta in order to fly together as a single combined particle. Another group at the Jefferson Lab uses similar energies as at the SPring-8 experiment, but their detection system covers a wider angular range, having hardly any coverage in the forward scattering region (which may be the region in which the pentaquark dominates). In addition, they also produce their gamma rays using the Bremsstrahlung method, for which the background level at lower energies is relatively high. Particle detections at very forward directions, and production of a gamma spectrum with low background at lower energies are unique features realised by the laser backscattering method, and therefore may explain some of the different results found by the different laboratories.
In order to try to support the existence of the pentaquark, there are two efforts. One is underway, and the other is being planned. The experiment underway is to repeat the prior experiment, but with a longer accumulation time to get counting statistics of at least three times better than in the prior experiment. The other effort is the construction of another beamline with a better detector (indeed, one that requires its own massive building separate from the SPring-8 building, and needs to be placed partially below ground level). This new detector will be able to collect a more complete angular range as well as the forward scattering region. With the wide region, the overlap with other experiments should allow for direct comparisons with data of other experimental groups. This detector is scheduled to arrive from Brookhaven in April or May, and the first results are expected early 2012. In addition to these efforts there are plans to combine the kaons with neutrons to form the pentaquark at the kaon beamline in J-Parc.
While the existence of this pentaquark will not severely affect daily life just yet, the story does provide us with a glimpse into the life at a very special SPring-8 beamline. More importantly, the physics established today will form the basis for your future and that of your descendants. High time, therefore, to catch up with these developments while you can.
(With thanks to dr. Schin Daté at SPring-8).
Leave a Reply